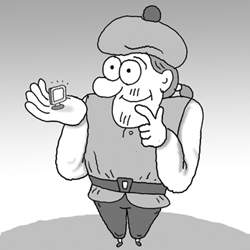
Most proteins, the chemical workhorse of the cell, are roughly 10 nanometers wide; a DNA molecule is about 2.5 nanometers.
Back in 1965, Intel co-founder Gordon Moore noticed that scientists were managing every year to double the data storage capacity of silicon chips used in computers. Now known as Moore's Law, this observation still holds true, though slightly extended - to roughly every 18 months. Engineers can now crunch some 30 million transistors onto a single microchip. However, miniaturization attempts will soon hit a wall, say experts, when the transistor will have become so tiny, designing it will be a messy, unreliable and highly costly adventure.
A partnership between electronics and nature may turn things around. Organic molecules already include tiny yet remarkably precise functional structures, such as the ribosome, which translates incoming messages encoded as DNA to build proteins. In microelectronics the aim would be to use organic molecules to enhance transistors, memory elements and other conventional components. Far more than just pushing back the impending miniaturization deadline, this merger may ultimately leave Moore’s Law in the dust.
Genes hooked up outside the cell
Shaped through time, biological cells are the ultimate engineering systems, able to perform the most advanced information-processing known as well as producing a wonderland of over 100,000 proteins. The cell pulls off these feats in a tiny setting that engineers can only dream of. How do its systems work? Might they be harnessed to build superfast computers or advance new biotechnologies?
In a step that might help address these questions, a Weizmann scientist has now designed the first synthetic circuit able to process genetic input to produce proteins. The circuit works on the principles of a conventional electrical circuit - that of a flashlight, for instance - but is constructed entirely of genes, proteins and other biological molecules. “Our goal was to determine whether an assembly of these components could be made to operate outside the context of a living cell,” says Dr. Roy Bar-Ziv of the Institute’s Materials and Interfaces Department who performed the work with Prof. Albert Libchaber and Dr. Vincent Noireaux of New York’s Rockefeller University.
The circuit inputs are genes “wired-up” such that the protein encoded for by one gene can either activate or depress the production of neighboring proteins. While other scientists have developed single-gene systems, this is this first time researchers have rigged up a multiple-gene circuit outside of the cell. Though rudimentary, this synthetic circuit offers an isolated and thus highly controllable environment in which to explore the workings of the cell, moreover it may represent the first step to streamlined protein production plants or advanced biocomputers. Unlike conventional computer systems, in which information is processed through a rigid digital 0-or-1, yes-or-no framework, biological networks are able to plod toward their goal using the multi-branched routes characteristic of parallel processing. This inherent property, researchers believe, might significantly fast-forward computer processing.
But this won’t happen any time soon. The system’s DNA-to-protein reactions can take an hour or more, and there is a delay until enough of the first material is produced to initiate the next stage. When too many stages are added to the sequence, the reactions tend to fizzle out as the available resources are used up.
The next step says Bar-Ziv, is to try and introduce circuitries of this sort into different materials. Once it is possible to create positive and negative feedback systems to turn things on and off, one could potentially design artificial circuits that mimic transistors, sensors, memory elements and clocks. “The gene is hardware and software all rolled up into one,” says Bar-Ziv. “Scientists are busy trying to invent self-replicating nanotechnology, but why not use what already exists?”
The goal: self-assembling transistors made of DNA
Merging a tiny sliver of bacterial DNA with carbon nanotubes and nearly 5 years of brainstorming, Institute scientists have created a self-assembling transistor that can interact with biological molecules.
The approach consists of a simple two-step self assembly process, enabling the simultaneous production of hundreds of transistors, the building blocks of electronics. The transistors can be designed to recognize a wide array of biological compounds - a feature that, in addition to computing and industrial applications, might advance the production of tiny sensors that would perform diagnostic tests in the body. The technique also offers the first-time possibility of modifying the properties of the transistor even after its production is complete.
The study, appearing in Chemical Physics Letters, was performed by a multidisciplinary team led by chemist Prof. Ron Naaman, physicist Dr. Dmitry Shvarts, biochemist Dr. Miron Hazani and doctoral students Dana Peled and Victor Sidorov.
The circuit consists of a carbon nanotube, a short segment of DNA and a gold-bottomed surface connected to gold electrodes. To achieve the unique property of self-assembly, the design takes advantage of the structure of DNA, which consists of two winding strands linked together by matching base pairs. One strand is connected to the nanotube, whereas its matching segment is bound to the gold surface. When the tube is introduced, its DNA strand naturally binds with the strand connected to the gold surface, creating a closed circuit. The result is a transistor that can be switched on and off by an electric current.
But biological molecules cannot conduct electricity, which is why nanowires such as carbon nanotubes enter the picture. The tubes are a natural candidate, due to their excellent electrical conductance and tiny dimensions.
Previous biocircuits have been characterized by low production yields and short-lived functionality, due to design flaws that caused rapid destruction of the biological molecules. The newly designed circuit allows for relatively large-scale production, and can operate for months on end. It also conducts an electric current that is 100 times stronger than those of previous devices.
To modify the transistor’s properties even after it is produced, the team introduces enzymes that function as a biological editing kit, cutting the DNA strands at certain points along its chain and adding on new sections.
“Many obstacles remain to producing marketable self-assembling transistors,” Naaman emphasizes. “But the dream is to create a sophisticated system that can sense, feel and react.”
Recollections of a pioneer
In the late 1940s John Bardeen, William Shockley and Walter Brattain of Bell Laboratories in New Jersey were trying to develop a semiconductor that would replace some of the vacuum tubes used in telecommunications. The tubes were impractical - they were energy inefficient, created heat and burned out rapidly.
The team was about to give up when a last attempt, using a purer substance, proved successful. Their discovery - the transistor - soon changed the world, becoming the building block for all modern electronics, including computers.
When asked if they had realized the importance of their discovery, for which they earned the 1956 Nobel prize in Physics, John Bardeen once replied: “No. When we invented the transistor it cost around fifty dollars to make, while the vacuum tube cost only a dollar and a half. We couldn’t imagine it ever offering a competitive alternative.” Today, around 100 million transistors can be produced for less than a dollar.
Organic molecules to fine-tune electronics
One challenge to incorporating biological compounds into electronics has been the difficulty of examining the electrical properties of organic molecules that are chemically connected to semiconductors and metals.
The layer of organic molecules used to cover semiconductors contains “pinholes” - small defects that are very difficult to detect but offer an easier route for electrons to pass through, thus radically altering conductance patterns. As a result, scientists taking electric measurements could not tell whether they were tracking the current’s passage through the molecule itself or through these pinholes.
Researchers at the Institute’s Materials and Interfaces Department skirted this problem by constructing a single layer of very short organic molecules, which they placed on a semiconductor. The monolayer was so thin that an electrical current directed through the semiconductor generally passed by the monolayer without interacting with it - making it irrelevant whether the electrons were passing through a molecule or a pinhole.
The team, including then graduate student Ayelet Vilan and Prof. David Cahen, also found that changing the organic molecules used led to predictable changes in electrical characteristics, meaning they could control the properties of the resulting electronic device. The molecules essentially served as “doormen,” determining the ease with which electrons passed through the device. Applying this understanding, the team (in collaboration with Prof. Abraham Shanzer of the Organic Chemistry Department), is now studying the use of novel organic molecules to fine-tune electronic devices.
Silicon real estate
Researchers at the Weizmann Institute and select teams worldwide have created transistor “switches” that are only a single molecule in size. A functional molecular transistor would represent the ultimate in electronic miniaturization. For perspective: if the conventional transistor were scaled up so that it occupied this page, a molecular transistor would be the dot on this i.
Prof. Abraham Shanzer of the Institute’s Organic Chemistry Department has designed such a single-molecule memory switch. The helix-structured organic molecules have upper and lower “pockets,” each of which binds electrically charged iron or copper ions. Using standard chemical reactions, the charge of the metal ion can be raised or lowered, causing it to jump between the two binding sites. These distinct states correspond to the zero or one of the binary code used in digital computers, thus fulfilling the basic requirement of a memory unit.
Key to implementing these switches is the ability to activate them, known as the “addressing” problem - which is where Prof. Israel Rubinstein of the Materials and Interfaces Department entered the picture. Rubinstein, together with Dr. Alexander Vaskevich and then doctoral student Gregory Kalyuzhny, demonstrated that the “switch” in these molecules could be activated by electrochemical means. They also showed that the molecules could be arranged as single molecular layers on electrode surfaces. Both characteristics are crucial to creating working memory components.
Further development of this concept could lead to digital circuitry containing millions of ON/OFF elements and dramatically more compact electronic devices. Nevertheless, significant challenges remain, including the ability to effectively link up these molecular switches or detect whether a particular molecular switch is ON or OFF.